Transmission lines: Important Types and Parameters
Read More About
Introduction
Transmission lines are a vital component of electrical power systems. They are used to transfer electrical energy over long distances from power plants to distribution networks or between different parts of a power grid. These lines are typically made of conductive materials, such as copper or aluminum, and are designed to transmit high voltage alternating current (AC) or direct current (DC).
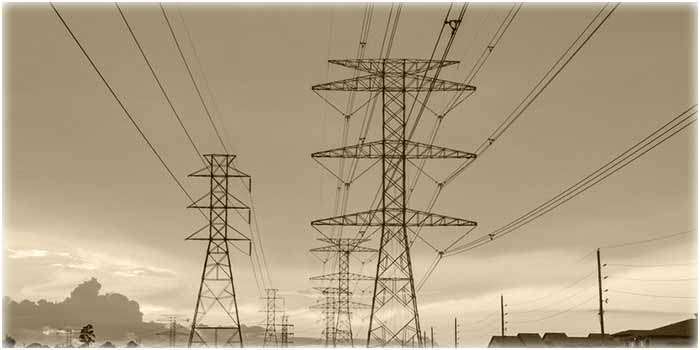
Here are some key aspects and characteristics of transmission lines:
Purpose: Transmission lines are used to transport electricity efficiently and reliably over long distances. They enable power generated at remote power stations to reach populated areas where electricity is needed.
Conductors: Transmission lines consist of conductors, which are typically made of stranded aluminum or copper wires. These conductors have a large cross-sectional area to minimize resistance and power losses during transmission.
Voltage Levels: Transmission lines operate at high voltages to reduce energy losses and increase the efficiency of power transmission. High-voltage transmission lines can operate at voltages ranging from a few tens of kilovolts (kV) to several hundreds of kilovolts.
AC and DC Transmission: Most transmission lines use alternating current (AC) due to its ability to be easily transformed to different voltage levels. However, direct current (DC) transmission or HVDC transmission is gaining popularity for long-distance transmission and connecting asynchronous power systems.
Insulation: Transmission lines require insulation to prevent leakage of electricity and ensure safety. Insulators made of materials like glass, porcelain, or composite polymers are used to support the conductors and keep them isolated from support structures.
Tower Structures: Transmission lines are supported by tall tower structures that provide mechanical support and keep the conductors at a safe distance from the ground. These towers are typically made of steel or concrete and are designed to withstand various weather conditions.
Capacitance and Inductance: Transmission lines exhibit capacitance and inductance due to the inherent properties of the conductors and their arrangement. These electrical characteristics can influence the performance and behavior of the transmission line, such as power factor and voltage regulation.
Power Losses: Transmission lines experience some level of power losses due to resistance, which leads to heat dissipation. Higher transmission voltages are used to minimize these losses, as power loss is proportional to the square of the current and resistance.
Line Constants: Transmission lines are characterized by parameters known as line constants, which include resistance, inductance, capacitance, and conductance. These constants determine the electrical behavior of the transmission line and are used in the analysis and design of power systems.
Challenges: Building and maintaining transmission lines involve several challenges, including right-of-way acquisition, environmental concerns, and system reliability. Additionally, power system engineers must consider factors like voltage regulation, reactive power compensation, and protection schemes to ensure efficient and reliable transmission.
Transmission lines play a crucial role in enabling the long-distance transmission of electrical power. They form the backbone of power grids, allowing electricity to be transmitted from generation sources to consumers across vast distances.
Types of Transmission lines
There are several types of transmission lines used in electrical power systems. The specific type of transmission line chosen depends on factors such as voltage level, power capacity, environmental conditions, and cost considerations. Here are some common types:
Overhead Transmission Lines
Overhead transmission lines are the most widely used type of transmission lines. They consist of conductors mounted on tall towers or poles. These lines are economical and relatively easy to construct and maintain. Overhead lines can be categorized based on the arrangement of conductors, such as:
a. Single Circuit Lines: Single circuit lines have one set of conductors per phase. They are commonly used in lower voltage transmission applications.
b. Double Circuit Lines: Double circuit lines have two sets of conductors per phase, typically arranged horizontally or vertically. They provide redundancy and are commonly used for higher voltage transmission.
c. Bundle Conductors: Bundle conductors consist of multiple smaller conductors bundled together in a single phase. This configuration increases the line’s capacity and reduces corona and radio interference.
Underground Transmission Cables
Underground transmission lines involve burying insulated cables underground. They are used in urban areas, environmentally sensitive regions, or locations where overhead lines are impractical. Underground cables offer advantages such as reduced visual impact, lower electromagnetic interference, and lower susceptibility to weather conditions. However, they are generally more expensive to install, maintain, and repair compared to overhead lines.
a. High Voltage Direct Current (HVDC) Cables: HVDC cables are used for long-distance HVDC transmission of direct current (DC). They are capable of transmitting high power over considerable distances with lower losses compared to AC lines.
Submarine Cables:
Submarine cables are used to transmit electricity across bodies of water, such as rivers, lakes, and seas. They are primarily used to connect offshore wind farms or to interconnect power systems across different land masses. Submarine cables are designed to be insulated and waterproof to ensure reliable transmission under water.
High-Temperature Low-Sag (HTLS) Conductors:
HTLS conductors are a specialized type of transmission line that uses composite materials or alloys to withstand higher temperatures and reduce conductor sag. These conductors allow for increased power transfer and can be used to upgrade existing transmission lines without requiring significant infrastructure modifications.
Superconducting Transmission Lines
Superconducting transmission lines utilize materials that exhibit zero electrical resistance at extremely low temperatures. These lines offer the advantage of near-zero power losses but require complex cooling systems, making them currently impractical for widespread use in power systems.
Each type of transmission line has its own advantages, limitations, and specific applications. Power system engineers carefully consider various factors to determine the most suitable type of transmission line for a given scenario, considering factors such as cost, technical feasibility, environmental impact, and system requirements.
Parameters of Transmission lines
Transmission lines are characterized by several parameters that describe their electrical behavior and performance. These parameters are used in the analysis, design, and operation of transmission line systems.
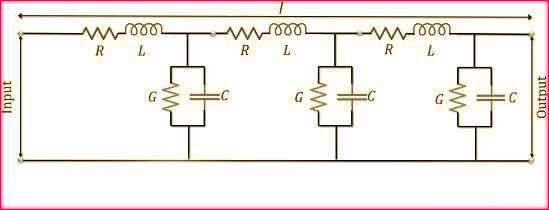
Here are the key parameters of transmission lines:
Resistance (R): Resistance represents the opposition to the flow of current in a transmission line. It is primarily caused by the resistance of the conductors. Resistance leads to power losses in the form of heat and is usually expressed in ohms per unit length (Ω/km or Ω/m).
Inductance (L): Inductance is the property of a transmission line that opposes changes in current flow. It is primarily due to the magnetic field generated by the current flowing through the conductors. Inductance affects the transmission line’s reactance and is measured in henries per unit length (H/km or H/m).
Capacitance (C): Capacitance is the ability of a transmission line to store electrical energy in an electric field. It exists between the conductors and the ground or between the conductors themselves. Capacitance affects the transmission line’s reactance and is typically measured in farads per unit length (F/km or F/m).
Conductance (G): Conductance represents the ease with which current can flow through a transmission line. It is the reciprocal of resistance and accounts for losses due to leakage currents and imperfections in insulation. Conductance is expressed in siemens per unit length (S/km or S/m).
Impedance (Z): Impedance is the total opposition offered by a transmission line to the flow of alternating current. It combines the effects of resistance (R) and reactance (X) and is a complex quantity, expressed in ohms (Ω).
Reactance (X): Reactance refers to the opposition offered by a transmission line to the flow of alternating current due to inductance (L) and capacitance (C). It is expressed in ohms and is typically separated into two components:
a. Inductive Reactance (XL): Inductive reactance arises from the inductance of the transmission line and is proportional to the frequency of the current. It is expressed in ohms.
b. Capacitive Reactance (XC): Capacitive reactance arises from the capacitance of the transmission line and is inversely proportional to the frequency of the current. It is expressed in ohms.
Velocity of Propagation (Vp): The velocity of propagation represents the speed at which electrical signals or energy travel along the transmission line. It is generally a fraction of the speed of light and depends on the characteristics of the line.
These parameters determine the electrical behavior of transmission lines, including characteristics such as impedance, voltage drop, power loss, and propagation delay. Power system engineers use these parameters to analyze and model transmission lines accurately, ensuring efficient and reliable power transmission.
Parameters Equations
The parameters of transmission lines can be calculated using various formulas and equations. Here are some commonly used formulas for calculating the parameters of transmission lines:
Resistance (R):
Resistance per unit length (R):
R = ρ * (L/A)
Where ρ is the resistivity of the conductor material, L is the length of the transmission line, and A is the cross-sectional area of the conductor.
Inductance (L):
Inductance per unit length (L):
L = (μ₀ * μᵣ * ln(b/a)) / (2π)
Where μ₀ is the permeability of free space, μᵣ is the relative permeability of the conductor material, b is the outer radius of the conductor, and a is the inner radius of the conductor.
Capacitance (C):
Capacitance per unit length (C):
C = (2π * ε₀ * εᵣ) / ln(b/a)
Where ε₀ is the permittivity of free space, εᵣ is the relative permittivity of the dielectric material between conductors, b is the outer radius of the conductor, and a is the inner radius of the conductor.
Impedance (Z):
Impedance per unit length (Z):
Z = sqrt((R + jωL) / (G + jωC))
Where R is the resistance per unit length, L is the inductance per unit length, G is the conductance per unit length, C is the capacitance per unit length, ω is the angular frequency (2πf), and j is the imaginary unit.
Propagation constant (γ):
γ = sqrt((R + jωL)(G + jωC))
Where R is the resistance per unit length, L is the inductance per unit length, G is the conductance per unit length, C is the capacitance per unit length, ω is the angular frequency (2πf), and j is the imaginary unit.
These formulas provide a basic understanding of how to calculate the parameters of transmission lines. However, it’s important to note that the calculations can become more complex when considering factors like skin effect, proximity effect, frequency-dependent properties, and line configuration.
In practical scenarios, sophisticated software tools and specialized techniques are often used for accurate parameter estimation and analysis of transmission lines.
Performance of transmission lines
The performance of transmission lines refers to their ability to effectively and efficiently transmit electrical power over long distances while meeting various technical requirements. Here are key aspects that define the performance of transmission lines:
Power Transfer Capacity: The power transfer capacity of a transmission line refers to the maximum amount of electrical power it can transmit without exceeding its thermal and voltage limits. Factors such as line parameters, conductor size, temperature, and cooling mechanisms determine the power transfer capacity.
Voltage Regulation: Transmission lines should maintain stable and acceptable voltage levels along their length to ensure proper operation of connected electrical equipment. Voltage regulation is achieved by considering factors such as line impedance, reactive power compensation, and control mechanisms to minimize voltage drop and maintain voltage within acceptable limits. It is given as below:
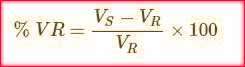
Where Vr is the receiving end voltage while the Vs is the sending end voltage. The sending end voltage are further calculated as below:

Line Losses: Transmission lines have inherent resistance, which results in power losses in the form of heat. Minimizing line losses is crucial for efficient power transmission. Higher voltage levels and optimized conductor sizes help reduce resistance and minimize power losses.
Reactive Power Compensation: Reactive power is required to maintain voltage levels and support the operation of inductive loads. Transmission lines may need reactive power compensation to control voltage fluctuations, improve power factor, and enhance system stability.
Devices such as shunt reactors, synchronous condensers, and static VAR compensators (SVCs) are used for reactive power control.
Fault Current Handling: Transmission lines must be designed to handle fault conditions, such as short circuits, and quickly isolate faulty sections to ensure minimal disruption to the power system. Protection schemes, including relays, circuit breakers, and fault current limiters, are employed to detect and isolate faults promptly.
Electromagnetic Interference: Transmission lines can generate electromagnetic fields that may interfere with nearby communication systems, especially in the radio frequency spectrum. Mitigation measures, such as proper line design, shielding, and grounding, are employed to reduce electromagnetic interference and ensure compatibility with other systems.
Reliability and Availability: Transmission lines play a critical role in maintaining the reliability and availability of power supply. They are expected to operate consistently and provide uninterrupted power transmission, even under adverse conditions such as severe weather, vegetation interference, and equipment failures.
Adequate maintenance, redundancy, and fault detection systems are essential to enhance the reliability of transmission lines.
Environmental Considerations: The environmental impact of transmission lines, including visual aesthetics, land use, and impact on wildlife, is taken into account during planning and design. Efforts are made to minimize the footprint of transmission lines and adopt environmentally friendly practices.
Efficient and reliable performance of transmission lines is crucial for the overall stability and functionality of electrical power systems. Power system engineers employ various techniques, technologies, and operational strategies to optimize the performance of transmission lines and ensure the secure and economical transmission of electrical power.
Efficiency of Transmission Line
The efficiency of a transmission line refers to the ratio of the power delivered at the load end to the power injected at the sending end. It represents how effectively the transmission line transfers electrical power from the source to the load, taking into account power losses along the line.
The efficiency of a transmission line can be calculated using the following formula:
Efficiency = (Power at Load End / Power at Sending End) * 100%

To calculate the power at the load end, you need to consider the voltage and current at the load end. If the load is purely resistive, you can use the formula:
Power at Load End = Voltage^2 / Load Resistance
If the load is a combination of resistance and reactance, you need to consider the complex power (apparent power) at the load end. The complex power is given by:
Complex Power at Load End = Voltage * Conjugate(Current at Load End)
The real power at the load end is the real part of the complex power, and the reactive power is the imaginary part. To calculate the power at the sending end, you can use similar formulas, considering the voltage and current at the sending end.
Efficiency is influenced by various factors, including the resistance of the transmission line, conductor material, length of the line, operating voltage, and power factor. Factors that increase resistance and reactance, such as long transmission distances or inadequate conductor size, can lead to higher power losses and reduced efficiency.
It’s important to note that transmission line efficiency is typically high, often exceeding 95%. However, it can vary based on the specific conditions and design parameters of the transmission line.
Power system engineers aim to minimize power losses and improve efficiency through proper line design, optimization of conductor size, voltage regulation, and reactive power compensation techniques.
Load Power Factor Impact on Efficiency
The load power factor has a significant impact on the efficiency of a transmission line. The power factor is a measure of the phase relationship between the voltage and current in an AC electrical system and is defined as the cosine of the angle between them. It indicates how effectively the electrical power is being used by the load.
The efficiency of a transmission line can be affected by the load power factor in the following ways:
Power Losses: Power losses in a transmission line are primarily caused by resistive components, such as the resistance of the conductors. These losses increase with higher currents flowing through the line.
When the load power factor is low (leading or lagging), it results in higher reactive power flow and increased line currents. This leads to higher resistive losses and reduces the overall efficiency of the transmission line.
Voltage Drop: Voltage drop occurs in a transmission line due to the resistance and reactance of the conductors. Higher currents resulting from a low power factor lead to larger voltage drops along the line. This can negatively impact the voltage profile and require higher voltage levels at the sending end to compensate for the drop. The higher voltage requirement may result in increased losses and reduced efficiency.
Line Loading: The load power factor affects the magnitude of the current flowing through the transmission line. Higher currents, as a result of a low power factor, increase the line loading. Overloading the line beyond its rated capacity can lead to higher resistive losses and potentially damage the line. Operating the line at higher currents reduces the overall efficiency.
To improve the efficiency of a transmission line, it is beneficial to operate the load with a power factor closer to unity (i.e., a power factor of 1). This reduces the reactive power flow, minimizes resistive losses, and helps maintain a better voltage profile along the line.
Power factor correction techniques, such as using capacitors or reactive power compensation devices, can be employed to improve the power factor and overall efficiency of the transmission line.
It is worth noting that power factor improvement may also have economic benefits, as utilities often charge customers for apparent power (kVA) rather than only for active power (kW). By improving the power factor, customers can reduce their apparent power demand and potentially lower their electricity bills.
End Condenser Method (Medium Transmission Line)
The End Condenser Method is a simplified method used for analyzing medium-length transmission lines. It provides an approximate solution to calculate voltage regulation and power flow in a transmission line without the need for complex mathematical calculations.
The method assumes that the distributed parameters (inductance and capacitance) of the transmission line are concentrated at the sending and receiving ends of the line.
Here’s a step-by-step explanation of the End Condenser Method for medium transmission lines:
Equivalent Circuit Representation: The medium transmission line is represented by an equivalent circuit consisting of a series impedance Z and two shunt admittances Yc, representing the concentrated capacitance at both ends.
Calculation of Shunt Admittance: The shunt admittance Yc can be approximated using the formula:
Yc = jωC / 2
Where ω is the angular frequency (2πf) and C is the total capacitance of the line.
Calculation of Series Impedance: The series impedance Z can be calculated by considering the resistance R and reactance X of the line:
Z = R + jX
Voltage Regulation: The voltage regulation (VR) of the transmission line can be calculated as:
VR = (Yc / (Yc + Ys)) * (1 – cosh((Yc + Ys) * l)) / sinh((Yc + Ys) * l)
Where Ys is the series admittance (Ys = 1 / Z) and l is the length of the transmission line.
Power Flow: The power flow (P) in the transmission line can be calculated using the formula:
P = V^2 * (Ys – Yc) * sinh((Yc + Ys) * l) / (2 * Ys * cosh((Yc + Ys) * l))
Where V is the sending end voltage.
It’s important to note that the End Condenser Method provides an approximate solution and is most accurate for lines with a length-to-wavelength ratio between 0.1 and 0.5.
For longer lines or higher accuracy requirements, more detailed analysis methods, such as the ABCD parameters or numerical techniques, should be used.
The End Condenser Method offers a simplified approach to analyze medium transmission lines and provides reasonable estimates for voltage regulation and power flow.
Nominal π Method (Medium Transmission Line)
The Nominal π (Pi) Method, also known as the Nominal π Model, is a widely used approximation method for analyzing medium-length transmission lines. It provides a simplified representation of the transmission line that facilitates calculations of voltage regulation, power flow, and other parameters.
In the Nominal π Method, the transmission line is represented by an equivalent circuit consisting of a π-shaped network. The model assumes that the distributed parameters of the transmission line, such as inductance and capacitance, are concentrated at the sending and receiving ends.
Here’s a step-by-step explanation of the Nominal π Method for medium transmission lines:
Equivalent Circuit Representation: The medium transmission line is represented by an equivalent π-shaped circuit. The π model consists of a series impedance Z and two shunt admittances Y, representing the concentrated capacitance at both ends.
Calculation of Shunt Admittance: The shunt admittance Y can be approximated using the formula:
Y = jωC / 2
Where ω is the angular frequency (2πf) and C is the total capacitance of the line.
Calculation of Series Impedance: The series impedance Z can be calculated by considering the resistance R and reactance X of the line:
Z = R + jX
Voltage Regulation: The voltage regulation (VR) of the transmission line can be calculated as:
VR = 2 * (Z + Y/2) * l * tanh((Z + Y/2) * l)
Where Z is the series impedance, Y is the shunt admittance, and l is the length of the transmission line.
Power Flow: The power flow (P) in the transmission line can be calculated using the formula:
P = V^2 * (Y – Y/2 * tanh((Z + Y/2) * l)) * sinh((Z + Y/2) * l)
Where V is the sending end voltage.
The Nominal π Method provides a simplified approach to analyze medium transmission lines and offers reasonable accuracy for lines with a length-to-wavelength ratio between 0.1 and 0.5.
For longer lines or higher accuracy requirements, more detailed analysis methods, such as the ABCD parameters or numerical techniques, should be considered.
Please note that the Nominal π Method is an approximation, and while it can provide useful estimates for voltage regulation and power flow, it may not capture all the complexities of the actual transmission line behavior.
ubscribe to our Newsletter “Electrical Insights Daily” to get the latest updates in Electrical Engineering. You can also Follow us LinkedIn to see our latest posts.
Frequently Asked Questions
What is the transmission line?
A transmission line is a structure or medium used to transport electrical energy from one point to another. It is commonly used to transmit high-voltage electricity over long distances, connecting power generation sources such as power plants to substations, which then distribute electricity to consumers.
What are the main components of a transmission line?
The main components of a transmission line include conductors, insulators, support structures, and associated equipment. The conductors carry the electrical current, while the insulators provide electrical insulation and support the conductors. Support structures, such as towers or poles, hold the conductors and insulators in place. Additional equipment includes transformers, switches, and protection devices.
What types of conductors are used in transmission lines?
Transmission lines commonly use overhead conductors made of aluminum or aluminum alloy, sometimes reinforced with steel strands to increase their strength and conductivity. These conductors are typically in the form of stranded wires or bundled conductors to increase the power-carrying capacity.
How are transmission lines classified?
Transmission lines can be classified based on their operating voltage levels. They are generally categorized as extra high voltage (EHV) lines for voltages above 345 kV, high voltage (HV) lines for voltages between 69 kV and 345 kV, and medium voltage (MV) lines for voltages between 1 kV and 69 kV. Additionally, transmission lines can be classified as overhead lines, underground cables, or submarine cables based on their installation method.
What are the advantages of overhead transmission lines?
Overhead transmission lines offer several advantages, including lower installation and maintenance costs compared to underground or submarine cables. They have higher power-carrying capacity, better heat dissipation, and are easier to inspect and repair. Overhead lines also have lower losses and can span long distances with fewer voltage drop issues.
What are the disadvantages of overhead transmission lines?
Overhead transmission lines have a few disadvantages. They are susceptible to weather conditions such as lightning strikes, strong winds, and ice formation, which can cause disruptions and outages. Visual impact and land requirements for constructing transmission line corridors are also considered disadvantages in certain areas. Additionally, overhead lines can pose environmental risks to birds and other wildlife.
What are the advantages of underground transmission cables?
Underground transmission cables offer advantages such as reduced visual impact and lower environmental impact compared to overhead lines. They are less susceptible to weather-related disruptions and have lower transmission losses. Underground cables also have a smaller footprint and can be installed in densely populated areas without significant land requirements.
How is the voltage level of a transmission line determined?
The voltage level of a transmission line is determined based on various factors, including the power generation capacity, the distance to be covered, the electrical load requirements, and regulatory standards. Higher voltage levels are used for long-distance transmission to minimize transmission losses, while lower voltage levels are employed for shorter distances or distribution networks.